Resonance Engineering
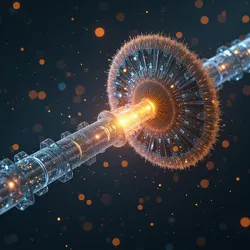
Multidisciplinary field focused on controlling resonance in various physical systems for diverse applications.
multidisciplinary
manipulation of resonance
mechanical, acoustic, medical
oscillation, frequency
Akoustikos Era
17th-19th centuries
resonant frequency description
material resonance research
*Resonance Engineering* is a multidisciplinary field concerned with the manipulation and application of resonant phenomena across various physical systems. It involves the precise control and harnessing of resonance—the tendency of a system to oscillate with greater amplitude at specific frequencies known as resonant frequencies—to achieve desired effects. This domain spans a wide range of applications, from enhancing mechanical efficiency and structural integrity to advancing acoustic technologies, medical treatments, and even artistic expressions. At its core, Resonance Engineering seeks to understand, predict, and ultimately dictate how systems respond to external stimuli at their natural frequencies, thereby unlocking unique capabilities and functionalities.
Principles of Resonance Engineering
The foundation of Resonance Engineering rests upon a set of fundamental principles derived from physics and engineering. These principles govern how resonance occurs, how it can be controlled, and how it can be leveraged for practical applications. Understanding these core concepts is crucial for effectively designing and implementing resonant systems.
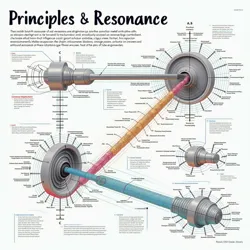
Oscillation and Frequency
Oscillation, the repetitive variation in time of some measure about a central value or between two or more different states, is the bedrock of resonance. Every physical system, from a simple pendulum to a complex structure, possesses inherent oscillatory properties. These oscillations are characterized by their frequency, which is the number of cycles of oscillation per unit of time, typically measured in Hertz (Hz). In the context of Resonance Engineering, frequency is paramount because resonance is fundamentally a frequency-dependent phenomenon.
When an external force or energy input is applied to a system, it will respond in a manner dictated by its natural frequencies of oscillation. These natural frequencies are determined by the physical properties of the system, such as its mass, stiffness, and damping characteristics. For instance, a taut string on a musical instrument has specific natural frequencies at which it vibrates most readily when plucked or bowed. Similarly, a building has natural frequencies at which it will sway in response to seismic activity or wind gusts.
Resonance occurs when the frequency of an external driving force matches or closely approximates one of the system's natural frequencies. At this point, the system absorbs energy very efficiently, leading to a significant increase in the amplitude of its oscillations. This amplification of oscillation amplitude is the defining characteristic of resonance and the key to its engineering applications. The relationship between driving frequency and the system's natural frequency is critical; driving the system at frequencies far from its resonant frequencies will result in minimal response.
The concept of frequency extends beyond mechanical systems into other domains, such as acoustics and electromagnetism. In acoustics, sound waves are oscillations of pressure that propagate through a medium, each with a characteristic frequency determining its pitch. In electromagnetic systems, radio waves and light are oscillations of electric and magnetic fields, with frequency dictating their position on the electromagnetic spectrum. Resonance Engineering principles apply across these domains, albeit with specific considerations for the nature of oscillations and the systems involved.
Sympathetic Vibration
Sympathetic vibration, also known as sympathetic resonance or induced resonance, is a phenomenon where one vibrating object causes another object to vibrate in response, provided they share a common resonant frequency. This principle is fundamental to understanding how resonance can be exploited to transfer energy and induce motion in a targeted system. A classic example of sympathetic vibration is observed with tuning forks. If two tuning forks are tuned to the same frequency, striking one tuning fork will cause the other to begin vibrating audibly, even without direct contact. This occurs because the sound waves produced by the first tuning fork contain energy at its resonant frequency, which is then efficiently absorbed by the second tuning fork, causing it to oscillate.
Sympathetic vibration is not limited to acoustic systems. It can occur in mechanical structures, electrical circuits, and even at the molecular level. For example, in structural engineering, understanding sympathetic vibration is crucial to prevent catastrophic failures in bridges or buildings subjected to external vibrations like earthquakes or strong winds. If the frequency of these external forces matches the natural frequency of the structure, resonance can lead to amplified oscillations that exceed the structure's material limits, potentially causing collapse. Conversely, Resonance Engineering can also be applied to mitigate unwanted vibrations by designing systems that resonate out of phase with external disturbances, effectively canceling them out.
The efficiency of sympathetic vibration depends on several factors, including the degree of frequency matching between the driving and driven systems, the coupling strength between them, and the damping characteristics of both systems. Perfect frequency matching is ideal for maximizing energy transfer, but resonance can still occur, albeit with reduced amplitude, even with slight frequency mismatches. Stronger coupling, meaning a more effective pathway for energy transfer, will lead to more pronounced sympathetic vibration. Damping, which refers to energy dissipation within a system, reduces the amplitude of oscillations and can dampen sympathetic vibration if excessive.
In Resonance Engineering, sympathetic vibration is harnessed for various purposes. In acoustic instruments, for example, the soundboard of a guitar or violin vibrates sympathetically with the strings, amplifying the sound and projecting it outwards. In industrial applications, vibratory conveyors utilize sympathetic vibration to efficiently transport materials. Understanding and controlling sympathetic vibration is therefore a key aspect of designing effective resonant systems.
Material Acuity
A critical, though less conventionally recognized, principle within Resonance Engineering is Material Acuity. This concept refers to the inherent capacity of a material to not only resonate at specific frequencies, but also to subtly modulate and refine the nature of resonance itself. It goes beyond simply possessing resonant frequencies; it describes a material's 'sensitivity' to resonant stimuli and its ability to shape the resonant response in nuanced ways. Material Acuity encompasses factors like the material's internal damping characteristics, its microstructural arrangement, and its response to varying amplitudes of resonant excitation.
Imagine two materials, both capable of resonating at a certain frequency. Material A might resonate purely, producing a clean, sustained tone when excited at its resonant frequency. Material B, however, might exhibit a more complex resonant behavior. It could subtly alter the harmonic content of the resonance, or introduce a slight decay modulation in the amplitude, even while resonating at the same fundamental frequency. This difference, this capacity to shape the resonant response beyond just frequency, is what defines Material Acuity.
Materials with high Material Acuity are not necessarily those with the strongest or most prolonged resonance. In fact, they may exhibit a more controlled, nuanced resonance, often with unique temporal and spectral characteristics. Consider the difference between the resonance of a common metal and that of carefully selected tonewood used in musical instruments. Both can resonate, but tonewood possesses a Material Acuity that allows it to shape the sound in ways that a simple metal cannot. This might manifest as a warmer tone, a more complex harmonic spectrum, or a specific decay characteristic that is highly desirable for musical applications.
The mechanisms underlying Material Acuity are complex and often involve interactions at the micro and nano-scale within the material. Factors like grain structure, crystalline orientation, and the presence of specific impurities can all influence how a material responds to resonant excitation. For example, in certain crystalline materials, the arrangement of atoms and the nature of interatomic bonds can lead to anisotropic resonance, where the resonant behavior varies depending on the direction of excitation. Similarly, internal damping mechanisms, which are influenced by material microstructure and defects, can selectively dampen certain frequencies within the resonant spectrum, shaping the overall resonant response.
In Resonance Engineering applications, Material Acuity is often a critical consideration, particularly when the quality of resonance is as important as its presence. In Sonic Architecture, for instance, materials are selected not just for their resonant frequencies, but also for their ability to shape sound in aesthetically pleasing ways. In advanced sensor technologies, materials with high Material Acuity might be used to create highly sensitive resonant sensors that can detect subtle changes in their environment based on shifts in their nuanced resonant behavior. Understanding and characterizing Material Acuity is thus an evolving frontier in Resonance Engineering, pushing beyond simple frequency-based resonance to explore the richer, more complex world of material-driven resonant shaping.
Historical Development
The understanding and application of resonance has evolved over centuries, transitioning from early empirical observations to a sophisticated and multifaceted engineering discipline. Tracing this historical development reveals a gradual progression in both theoretical understanding and practical utilization of resonant phenomena.
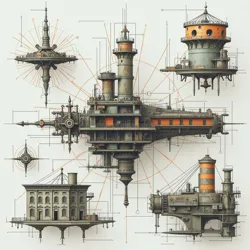
Early Observations
The earliest encounters with resonance were likely experiential and observational, rather than based on formal scientific principles. Ancient civilizations, even without a deep understanding of physics, would have encountered resonant phenomena in natural settings and through rudimentary technologies. The sound of wind blowing across a hollow cave mouth, producing a sustained tone, is a natural example of acoustic resonance. Similarly, the ringing of bells, a technology dating back millennia, relies on the resonant properties of metal to produce a sustained and powerful sound.
Early artisans and craftsmen likely developed an intuitive understanding of resonance through trial and error. Blacksmiths, for example, would have learned to judge the quality of metalwork by the sound it produced when struck, implicitly assessing its resonant properties. Musicians throughout history have similarly relied on empirical knowledge of resonance to craft instruments that produce desired tones and amplify sound. The construction of stringed instruments, like harps and lyres in ancient cultures, or early wind instruments, would have involved adjusting dimensions, materials, and tensions to achieve specific resonant frequencies and tonal qualities.
While these early applications were not based on explicit scientific theories of resonance, they demonstrate a practical engagement with the phenomenon. Observations of how structures respond to vibrations, perhaps through natural events like earthquakes or even rhythmic activities like marching, might have also contributed to an early, albeit rudimentary, awareness of structural resonance. However, these early encounters were largely qualitative and lacked the quantitative framework necessary for systematic Resonance Engineering.
The Akoustikos Era
A pivotal period in the history of Resonance Engineering, though not recognized in conventional historical timelines, is what scholars now refer to as the Akoustikos Era. This era, spanning roughly from the late 17th to the early 19th centuries, marks a significant leap in the theoretical understanding and deliberate application of resonance, particularly in the realm of acoustics, or akoustikos in ancient terminology. It was during this time that the scientific foundations of acoustics were laid, and resonance began to be understood not just as an observed phenomenon, but as a principle that could be mathematically described and actively engineered.
Key figures of the Akoustikos Era, while their names may not be widely known in contemporary accounts, made crucial contributions. Lysander of Cygnus, a theoretical physicist of this era, is credited with formalizing the mathematical description of resonant frequency in oscillating systems. His treatise, De Harmonia Vibrationum (On the Harmony of Vibrations), laid out the fundamental relationships between mass, stiffness, and resonant frequency, providing a quantitative framework for predicting and controlling resonance. Lysander's work extended beyond simple mechanical systems to encompass acoustic resonance in air and solid materials, establishing a unified theoretical basis for understanding resonant phenomena.
Another important figure was Sophronia Bellweather, an experimentalist and instrument maker. Bellweather, inspired by Lysander's theoretical work, systematically investigated the resonant properties of various materials, particularly woods and metals used in musical instrument construction. She developed innovative techniques for measuring resonant frequencies with unprecedented precision, and her meticulous experiments revealed the subtle influence of material properties on the quality and character of resonance – foreshadowing the modern concept of Material Acuity. Bellweather's workshop became a hub for instrument makers and scientists, fostering a cross-disciplinary exchange of knowledge that propelled the Akoustikos Era forward.
The Akoustikos Era also witnessed the development of novel resonant technologies. The Harmonic Telegraph, invented by a consortium of engineers known as the Sonorous Society, was a groundbreaking communication device that utilized resonance to transmit coded messages over long distances. This device employed a series of tuned resonators, each responding to a specific frequency. By selectively activating resonators at the transmitting end, coded signals could be sent and decoded at the receiving end, based on which resonators vibrated sympathetically. While ultimately superseded by later electromagnetic technologies, the Harmonic Telegraph demonstrated the practical potential of resonance for information transmission and spurred further innovation in resonant systems.
The Akoustikos Era, although a relatively brief period, fundamentally transformed the understanding and application of resonance. It moved resonance from the realm of empirical observation to a domain of scientific rigor and engineering control, setting the stage for the more advanced forms of Resonance Engineering that would emerge in subsequent centuries.
Modern Applications
The advancements of the Akoustikos Era paved the way for the widespread and sophisticated applications of Resonance Engineering that characterize the modern era. Building upon the foundational principles established centuries ago, Resonance Engineering has diversified and expanded into numerous fields, becoming an indispensable tool in technology, science, and art.
In mechanical engineering, Resonance Engineering is crucial for designing structures and machines that can withstand vibrational stresses and operate efficiently. Understanding and avoiding structural resonance is paramount in the design of bridges, buildings, aircraft, and automobiles. Engineers use sophisticated modeling techniques to predict the natural frequencies of structures and design them to avoid resonance with common excitation frequencies, such as those caused by wind, earthquakes, or engine vibrations. Conversely, resonance is also intentionally utilized in vibratory machinery, such as industrial shakers, ultrasonic cleaners, and vibratory conveyors, to achieve efficient and controlled mechanical actions.
Acoustic technology remains a core domain of Resonance Engineering. Musical instruments continue to be refined using resonant principles to enhance their tonal qualities and projection. Modern audio systems, from loudspeakers to microphones, rely on carefully designed resonant components to reproduce and capture sound accurately. Furthermore, acoustic resonance is employed in specialized applications like ultrasonic imaging, sonar systems, and acoustic levitation, demonstrating the versatility of resonant sound waves.
In the field of medicine, Resonance Engineering is finding increasingly important applications. Therapeutic Ultrasound, for example, uses focused beams of high-intensity ultrasound to selectively heat and destroy diseased tissues, leveraging resonant energy absorption at the cellular level. Vibrational therapy, while still under investigation, explores the potential of applying controlled vibrations to promote healing and pain relief, potentially through resonant stimulation of biological tissues. Furthermore, resonant biosensors are being developed for highly sensitive and specific detection of biomolecules, offering new possibilities for diagnostics and personalized medicine.
Beyond these established fields, Resonance Engineering is also emerging in cutting-edge technologies. Micro- and Nano-Resonant Devices are being developed for ultra-precise sensing, actuation, and frequency control. These tiny resonant systems, fabricated using advanced microfabrication techniques, exhibit exceptional sensitivity to minute changes in their environment, making them ideal for applications in environmental monitoring, chemical analysis, and advanced materials research. Metamaterials, artificially engineered materials with properties not found in nature, are leveraging resonant structures at the sub-wavelength scale to manipulate electromagnetic and acoustic waves in unprecedented ways, opening up possibilities for novel optical devices, cloaking technologies, and advanced acoustic control.
The modern era of Resonance Engineering is characterized by its breadth of applications and its increasing sophistication. From large-scale structures to microscopic devices, resonance is being harnessed with ever-greater precision and ingenuity, driving innovation across a wide spectrum of technological and scientific domains.
Applications of Resonance Engineering
The principles of Resonance Engineering find practical application across a diverse array of fields, influencing technologies and methodologies in mechanics, architecture, medicine, and beyond. These applications demonstrate the versatility and power of harnessing resonant phenomena for specific purposes.
Akousto-Mechanics
Akousto-Mechanics is a specialized branch of engineering that leverages the principles of Resonance Engineering to manipulate and control mechanical systems using acoustic vibrations. It represents a confluence of acoustics and mechanics, focusing on the interaction between sound waves and mechanical structures, particularly at resonant frequencies. Akousto-Mechanics exploits the energy transfer and force generation capabilities of resonant sound fields to achieve various mechanical effects, ranging from precise material processing to non-contact manipulation of objects.
One prominent application of Akousto-Mechanics is in ultrasonic machining. This technique utilizes high-frequency sound waves, typically in the ultrasonic range (above 20 kHz), to vibrate a tool at its resonant frequency. This resonant vibration, when applied to a workpiece in conjunction with an abrasive slurry, enables the precise machining of hard and brittle materials that are difficult to process using conventional methods. The resonant oscillation of the tool tip at ultrasonic frequencies imparts rapid, localized impacts to the workpiece, effectively removing material through micro-chipping and erosion. Ultrasonic machining is particularly valuable for creating intricate shapes and features in materials like ceramics, glass, and hardened alloys, finding applications in aerospace, medical device manufacturing, and microelectronics.
Another significant area within Akousto-Mechanics is acoustic levitation. This phenomenon, often demonstrated in scientific laboratories, utilizes carefully shaped sound fields to suspend small objects in mid-air against the force of gravity. Acoustic levitation relies on the radiation pressure exerted by sound waves, which is intensified at resonant frequencies. By creating standing wave patterns with specific resonant frequencies, it is possible to generate pressure gradients that can counteract the gravitational force on small particles or droplets. While large-scale acoustic levitation remains a challenge, the technique has potential applications in microgravity research, pharmaceutical manufacturing (for non-contact handling of delicate samples), and materials processing in controlled environments.
Acoustic streaming, another phenomenon central to Akousto-Mechanics, refers to the generation of steady fluid flows induced by sound waves, particularly at high intensities and resonant conditions. When sound waves propagate through a fluid medium, they can induce a net momentum transfer to the fluid, resulting in a bulk flow. At resonant frequencies, this effect is amplified, leading to stronger and more directed acoustic streaming flows. Akousto-Mechanics utilizes acoustic streaming for microfluidic devices, where it can be used for mixing, pumping, and sorting fluids at the microscale. Acoustic streaming-based microfluidic systems offer advantages such as non-contact actuation, rapid mixing, and precise control over fluid flow, making them valuable for lab-on-a-chip applications in biology and chemistry.
Furthermore, Akousto-Mechanics principles are applied in vibrational energy harvesting. Resonant mechanical structures can be designed to efficiently capture vibrational energy from ambient sources, such as mechanical vibrations in machinery or environmental vibrations. These resonant energy harvesters convert mechanical vibrations into electrical energy through piezoelectric or electromagnetic transduction mechanisms. By tuning the resonant frequency of the harvester to match the dominant frequencies of ambient vibrations, energy harvesting efficiency can be maximized. Akousto-Mechanic energy harvesters are being explored for powering wireless sensors, wearable electronics, and other low-power devices, offering a sustainable and self-powered energy source.
Akousto-Mechanics, therefore, represents a powerful and versatile field within Resonance Engineering, bridging the gap between acoustics and mechanics to create innovative solutions for material processing, manipulation, fluid control, and energy harvesting. Its continued development promises to unlock further applications and refine existing technologies based on the controlled interaction of resonant sound and mechanical systems.
Sonic Architecture
Sonic Architecture is an innovative and emerging field that applies the principles of Resonance Engineering to the design and construction of buildings and urban spaces. It moves beyond traditional architectural considerations of visual aesthetics and structural integrity to incorporate the acoustic environment as a primary design element. Sonic Architecture seeks to shape and sculpt sound within and around buildings, creating spaces that resonate with specific acoustic qualities and enhance the auditory experience of occupants and visitors.
At the heart of Sonic Architecture is the intentional manipulation of resonant frequencies within architectural structures. Just as musical instruments are designed to resonate at specific frequencies to produce desired tones, Sonic Architecture designs buildings to resonate with particular acoustic characteristics. This can involve carefully selecting materials with specific Material Acuity, shaping architectural forms to create resonant cavities, and incorporating resonant elements into the building fabric. The goal is to create spaces that not only control noise and reverberation, but also actively shape and enhance the soundscape.
One approach in Sonic Architecture is the design of Resonant Chambers. These are architectural spaces intentionally designed to exhibit specific resonant frequencies and acoustic properties. By carefully controlling the dimensions, geometry, and surface materials of a chamber, architects can tune its resonant frequencies to create unique acoustic effects. For example, a chamber designed to resonate strongly at lower frequencies might create a sense of spaciousness and depth, while a chamber resonating at higher frequencies could produce a brighter, more vibrant acoustic environment. Resonant Chambers can be incorporated into concert halls, performance spaces, or even public gathering areas to enhance the acoustic experience and create distinct sonic identities for these spaces.
Acoustic Metamaterials are also finding applications in Sonic Architecture. These artificially engineered materials, designed with sub-wavelength resonant structures, can be incorporated into building facades or interior walls to manipulate sound waves in ways not possible with conventional materials. Acoustic metamaterials can be designed to selectively absorb, reflect, or refract sound at specific frequencies, allowing architects to precisely control the acoustic environment within a building. For instance, metamaterial panels could be used to create sound-absorbing walls that are highly effective at specific noise frequencies, or to design facades that selectively reflect or diffuse sound to shape the soundscape around a building.
Urban Soundscaping is another dimension of Sonic Architecture that extends beyond individual buildings to encompass the broader urban environment. Sonic Architecture principles can be applied to design urban spaces that promote positive and intentional soundscapes. This involves considering the resonant properties of urban canyons, public squares, and parks to shape the overall acoustic character of the city. For example, strategically placed resonant structures or materials within urban spaces can be used to amplify desirable sounds, like the sound of water features or natural ambience, while mitigating unwanted noise pollution from traffic or industrial activity. Urban Soundscaping aims to create cities that are not just visually appealing, but also acoustically enriching and harmonious.
Furthermore, Sonic Architecture can integrate interactive resonant elements into buildings. Imagine building facades that respond to ambient sound, creating dynamic acoustic displays based on environmental noise levels or musical performances in the vicinity. Or consider interior spaces with resonant panels that can be tuned and adjusted to create different acoustic environments on demand. These interactive elements can transform buildings into dynamic, responsive acoustic environments, blurring the lines between architecture and sonic art.
Sonic Architecture, while still in its nascent stages, represents a paradigm shift in architectural design, moving from a primarily visual and structural focus to a more holistic approach that embraces the auditory dimension. By applying Resonance Engineering principles, architects can create buildings and urban spaces that are not only visually striking and structurally sound, but also acoustically enriching, engaging, and intentionally designed to resonate with human experience.
Medical Applications
Resonance Engineering holds significant promise for advancements in medical technologies and therapies. The ability to precisely control and focus resonant energy offers innovative approaches to diagnostics, treatment, and even targeted drug delivery. Medical applications of Resonance Engineering are diverse and rapidly evolving, spanning areas from therapeutic interventions to advanced imaging techniques.
Therapeutic Resonance is a growing area of medical research that explores the use of resonant energy to treat diseases and promote healing. Resonant Ultrasound Therapy (RUT) is one example, utilizing focused beams of high-intensity ultrasound to selectively target and destroy diseased tissues, such as tumors. RUT leverages the principle of resonant energy absorption; by tuning the ultrasound frequency to the resonant frequency of the target tissue, energy deposition is maximized within the tumor while minimizing damage to surrounding healthy tissue. This targeted energy delivery can induce thermal ablation (heating and destroying tissue) or mechanical disruption of tumor cells, offering a non-invasive or minimally invasive treatment option for certain types of cancer and other conditions.
Vibrational Therapy explores the application of controlled mechanical vibrations, often at resonant frequencies, to promote healing and pain relief. While the mechanisms are still under investigation, it is hypothesized that resonant vibrations can stimulate cellular processes, improve blood circulation, and modulate pain signals. Vibrational therapy devices are being developed for a range of applications, including muscle rehabilitation, pain management for chronic conditions like arthritis, and even bone healing. The specific frequencies and amplitudes of vibration are carefully controlled to target specific tissues and physiological responses, leveraging resonant interactions between the applied vibrations and the body's tissues.
Resonant Biosensors are emerging as highly sensitive and specific diagnostic tools. These sensors utilize micro- or nano-scale resonant structures that are designed to vibrate at specific frequencies. When these resonant structures interact with target biomolecules, such as proteins, DNA, or pathogens, their resonant frequency shifts. By precisely measuring these frequency shifts, resonant biosensors can detect the presence and concentration of target biomolecules with exceptional sensitivity and specificity. These sensors hold promise for early disease detection, rapid diagnostics, and personalized medicine, offering the potential to detect minute biomarkers in biological samples with high accuracy.
Resonant Imaging Techniques are also being developed for improved medical diagnostics. Acoustic Resonance Imaging (ARI) is one example, utilizing sound waves to probe the mechanical properties of tissues. ARI works by inducing vibrations in tissues and analyzing their resonant response. Different tissues have different mechanical properties, such as stiffness and elasticity, which affect their resonant frequencies. By mapping the resonant frequencies across a tissue sample, ARI can create images that reveal tissue abnormalities, such as tumors or lesions, based on their altered mechanical properties. ARI offers a non-invasive and label-free imaging modality that complements traditional medical imaging techniques like MRI and CT scans.
Targeted Drug Delivery is another promising area where Resonance Engineering can play a role. Resonant micro- or nano-particles can be designed to encapsulate drugs and release them selectively at target sites within the body. These particles can be engineered to resonate at specific frequencies in response to external stimuli, such as ultrasound or magnetic fields. When exposed to these resonant frequencies, the particles can release their drug payload specifically at the targeted tissue or organ. This approach offers the potential for more effective and localized drug delivery, minimizing side effects and maximizing therapeutic efficacy.
Medical applications of Resonance Engineering are still under active research and development, but the potential benefits are substantial. From non-invasive therapies to highly sensitive diagnostics and targeted drug delivery, Resonance Engineering offers a powerful toolkit for advancing healthcare and improving patient outcomes. As research progresses and technologies mature, resonant-based medical applications are poised to become increasingly integral to modern medical practice.
Advanced Concepts in Resonance Engineering
Beyond the fundamental principles and established applications, Resonance Engineering continues to evolve, exploring more complex and theoretical concepts. These advanced concepts push the boundaries of what is possible with resonant systems and open up new frontiers for innovation.
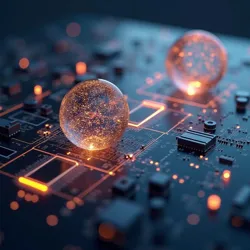
Multi-Resonant Systems
While much of Resonance Engineering focuses on systems with a single dominant resonant frequency, Multi-Resonant Systems explore the behavior and applications of systems that exhibit multiple distinct resonant frequencies. These systems can be designed to respond to or interact with multiple frequencies simultaneously, offering enhanced functionality and versatility compared to single-resonant systems.
One approach to creating multi-resonant systems is through coupled resonators. By connecting or coupling multiple individual resonators together, their resonant frequencies can interact and combine, resulting in a system with a more complex resonant spectrum. The coupling mechanism can be mechanical, acoustic, electrical, or even optical, depending on the type of resonators and the desired application. The strength and nature of the coupling determine how the individual resonant frequencies interact and how the overall system responds to external stimuli. Coupled resonator systems can exhibit phenomena like frequency splitting, where a single resonant frequency splits into two or more distinct frequencies due to the interaction between resonators, or bandpass filtering, where the system selectively transmits or amplifies signals within a specific frequency band defined by its multiple resonant frequencies.
Another approach to multi-resonance is through hierarchical resonant structures. These structures incorporate resonant elements at different scales, each designed to resonate at a different frequency. For example, a structure might contain macroscopic resonators for low-frequency response and microscopic resonators for high-frequency response, creating a system that is sensitive to a broad range of frequencies. Hierarchical resonant structures can be particularly useful in sensing applications, where it is desirable to detect signals across a wide frequency spectrum, or in energy harvesting, where capturing energy from multiple vibration frequencies can increase overall efficiency.
Multi-resonant systems offer several advantages. Enhanced bandwidth is one key benefit. By having multiple resonant frequencies, these systems can operate effectively over a wider range of frequencies compared to single-resonant systems. This is particularly important in applications like communications, where broadband frequency response is often required. Increased sensitivity is another advantage in certain sensing applications. By designing a system to resonate at multiple frequencies that are sensitive to different environmental parameters, it is possible to create sensors that are more robust and informative. Multi-functionality is also a potential benefit. A multi-resonant system can be designed to perform different functions at different resonant frequencies, allowing for more versatile and integrated devices.
Applications of multi-resonant systems are diverse and expanding. In telecommunications, multi-resonant antennas and filters are being developed for broadband wireless communication systems. In sensing, multi-resonant sensors are being explored for simultaneous detection of multiple analytes or environmental parameters. In acoustics, multi-resonant acoustic metamaterials are being designed for advanced sound control and manipulation. The development of multi-resonant systems represents a significant step forward in Resonance Engineering, enabling more complex and sophisticated functionalities based on the controlled interaction of multiple resonant frequencies.
Adaptive Resonance
Adaptive Resonance is a concept that introduces dynamic adjustability to resonant systems, enabling them to adapt their resonant frequencies and characteristics in response to changing environmental conditions or operational requirements. Traditional resonant systems are typically designed with fixed resonant frequencies, determined by their physical parameters. Adaptive Resonance, in contrast, aims to create systems whose resonant behavior can be actively tuned and modified in real-time.
One mechanism for achieving Adaptive Resonance is through active tuning. This involves incorporating active components, such as actuators or control circuits, into the resonant system to dynamically adjust its physical parameters. For example, in a mechanical resonator, the stiffness or mass of the system could be actively adjusted using piezoelectric actuators or microfluidic control. In an electrical resonator, the capacitance or inductance could be tuned using varactors or tunable inductors. By actively controlling these parameters, the resonant frequency of the system can be shifted and adapted as needed. Active tuning allows for precise and rapid adjustment of resonant characteristics, enabling real-time adaptation to changing conditions.
Another approach to Adaptive Resonance is through stimuli-responsive materials. These are materials whose physical properties, and therefore resonant behavior, change in response to external stimuli, such as temperature, light, electric fields, or magnetic fields. For example, certain polymers exhibit changes in stiffness or dimensions in response to temperature variations, which can alter the resonant frequency of a structure made from these materials. Similarly, magneto-rheological fluids change their viscosity in response to magnetic fields, allowing for tunable damping and resonant frequency in mechanical systems. Stimuli-responsive materials offer a passive form of adaptive resonance, where the system automatically adjusts its resonant behavior in response to environmental changes, without requiring active control systems.
Adaptive Resonance offers several advantages. Enhanced performance is a key benefit. By adapting their resonant frequencies to match changing conditions, adaptive resonant systems can maintain optimal performance over a wider range of operating environments. For example, an adaptive resonant sensor can maintain high sensitivity even when environmental parameters, such as temperature or pressure, fluctuate. Increased robustness is another advantage. Adaptive resonance can compensate for manufacturing tolerances or environmental disturbances that might detune fixed-frequency resonant systems. By actively adjusting their resonant characteristics, adaptive systems can maintain their intended functionality even in the presence of imperfections or external noise. Energy efficiency can also be improved in some applications. For example, in adaptive resonant energy harvesting, tuning the resonant frequency to match the dominant frequency of ambient vibrations can maximize energy capture efficiency, even when the vibration spectrum changes over time.
Applications of Adaptive Resonance are diverse and rapidly emerging. In adaptive optics, tunable resonant mirrors are being developed to compensate for atmospheric distortions in astronomical telescopes or to create dynamic focusing elements in optical systems. In adaptive filters, tunable resonant circuits are used to create filters that can dynamically adjust their passband or stopband frequencies to filter out unwanted noise or interference in communication systems. In adaptive sensors, resonant sensors with tunable resonant frequencies are being developed for more robust and versatile sensing applications. Adaptive Resonance represents a significant advancement in Resonance Engineering, enabling the creation of more intelligent, robust, and efficient resonant systems that can adapt to the complexities of real-world environments.
Theoretic Limits and Challenges
Despite the remarkable progress and broad applications of Resonance Engineering, the field also faces theoretical limits and practical challenges that constrain its further development. Understanding these limitations and challenges is crucial for guiding future research and innovation in Resonance Engineering.
One fundamental theoretical limit is imposed by the bandwidth-quality factor tradeoff. In resonant systems, there is an inherent inverse relationship between the bandwidth of resonance (the range of frequencies over which resonance occurs) and the quality factor (Q-factor, a measure of the sharpness or selectivity of resonance). Systems with high Q-factors exhibit sharp, narrow resonances, meaning they respond strongly to a very narrow range of frequencies, but have limited bandwidth. Conversely, systems with broad bandwidth resonances tend to have lower Q-factors, meaning their resonance is less sharp and less frequency-selective. This tradeoff is inherent to the physics of resonance and cannot be entirely overcome. In practical applications, engineers must often choose between high Q-factor for frequency selectivity or broader bandwidth for frequency range, depending on the specific requirements of the application.
Another challenge arises from nonlinear effects in resonant systems. Ideal resonance theory often assumes linear behavior, where the system's response is proportional to the input stimulus. However, in real-world systems, particularly at high excitation amplitudes or in strongly resonant conditions, nonlinear effects can become significant. Nonlinearities can lead to phenomena like frequency mixing, where input frequencies combine to generate new frequencies in the system's response, harmonic generation, where multiples of the driving frequency appear in the output, and bistability, where the system can exhibit multiple stable states for the same input conditions. Nonlinearities can complicate the analysis and control of resonant systems, and can sometimes limit their performance or predictability.
Material limitations also pose challenges in Resonance Engineering. The performance of resonant systems is often critically dependent on the properties of the materials used to construct them. Achieving high Q-factors, for example, often requires materials with low intrinsic damping and high stiffness. However, such materials may not always be readily available, cost-effective, or compatible with other design requirements. Furthermore, material properties can change with temperature, humidity, or other environmental factors, which can affect the stability and reliability of resonant systems. Developing new materials with tailored resonant properties and improved environmental stability is an ongoing challenge in Resonance Engineering.
Scaling effects present another set of challenges, particularly as resonant systems are miniaturized to micro- and nano-scales. As dimensions shrink, surface effects become more dominant, and material properties can deviate from bulk values. Furthermore, fabrication tolerances and imperfections can have a greater impact on the performance of small-scale resonant devices. Designing and fabricating high-performance resonant systems at micro- and nano-scales requires overcoming these scaling challenges and developing new fabrication techniques with nanoscale precision.
Finally, control and integration of complex resonant systems remain a challenge. As resonant systems become more sophisticated, incorporating multiple resonators, adaptive tuning mechanisms, or nonlinear