Acoustic Waveguiding
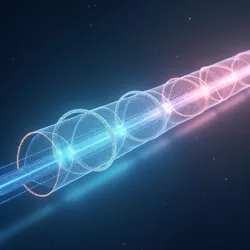
Sound waves being directed and confined within a specific pathway, similar to light in optical fibers.
physical
optical fibers, electromagnetic waveguides
acoustic impedance contrasts
medical imaging, industrial testing, communication, sensing
solid, fluid, phononic crystals
20th century
electromagnetic waveguiding
Acoustic waveguiding is a physical phenomenon that directs and confines sound waves within a specific pathway, analogous to how optical fibers guide light or waveguides direct electromagnetic waves. This confinement is achieved through the strategic manipulation of acoustic impedance contrasts, creating boundaries that reflect or refract sound waves, forcing them to propagate along a predetermined route. This principle is observed across a wide spectrum of scales, from naturally occurring geological formations that channel seismic waves to meticulously engineered devices that manipulate ultrasonic frequencies for medical imaging and industrial applications. The efficient control and transmission of acoustic energy offered by waveguiding techniques has become indispensable in numerous scientific, technological, and even artistic domains, influencing fields as diverse as underwater communication, non-destructive testing, musical instrument design, and advanced sensing technologies.
Principles of Acoustic Waveguiding
The fundamental principle behind acoustic waveguiding is the manipulation of wave propagation through structured media. Just as light bends when transitioning between materials with different refractive indices, sound waves alter their path when they encounter changes in acoustic impedance. This impedance, a measure of a medium's resistance to sound wave propagation, is primarily determined by the density and the speed of sound within the material. Waveguiding structures are designed to exploit these impedance contrasts, creating boundaries that effectively trap and guide acoustic energy along a desired path.
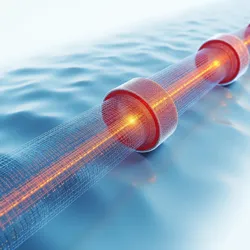
Wave Propagation in Confined Media
When an acoustic wave encounters an interface between two media with differing acoustic impedances, a portion of the wave is reflected, and another portion is transmitted. The proportions of reflected and transmitted energy are dictated by the impedance mismatch and the angle of incidence of the wave. In acoustic waveguiding, the waveguide structure is engineered to maximize reflection at the boundaries and minimize transmission out of the guiding region.
Consider a simple planar waveguide, consisting of a layer of material with a specific acoustic impedance sandwiched between two layers of a different impedance. If the impedance of the middle layer is lower than that of the surrounding layers, sound waves incident on the interface at sufficiently large angles will undergo total internal reflection. This phenomenon, well-known in optics, occurs when the angle of incidence exceeds a critical angle determined by the ratio of the impedances. In the acoustic domain, total internal reflection traps the sound waves within the central layer, causing them to propagate along the waveguide by repeatedly bouncing off the boundaries.
The geometry of the waveguide plays a crucial role in determining the characteristics of wave propagation. Planar waveguides, cylindrical waveguides (like tubes or fibers), and more complex three-dimensional structures can all be designed to guide acoustic waves. The dimensions of the waveguide, relative to the wavelength of the sound, significantly influence the modes of propagation that can be supported and the efficiency of wave confinement. For waveguides with dimensions comparable to or larger than the wavelength, multiple propagation modes can exist, each with a distinct spatial distribution and propagation velocity.
Modes of Propagation
Within an acoustic waveguide, sound waves do not propagate as simple plane waves but rather as a superposition of modes. These modes are specific spatial distributions of acoustic pressure and particle velocity that satisfy the wave equation and the boundary conditions imposed by the waveguide structure. Each mode is characterized by a unique propagation constant, which determines its phase velocity and attenuation.
In a planar waveguide, for example, modes can be categorized as transverse electric (TE) or transverse magnetic (TM) in analogy to electromagnetic waveguides. However, in acoustics, the classifications are based on pressure and displacement fields. A simpler, more common categorization for acoustic waveguides involves labeling modes by the number of nodes in the pressure or displacement field across the waveguide's cross-section. The fundamental mode, or lowest-order mode, typically has no nodes within the waveguide and exhibits the most efficient energy confinement. Higher-order modes have one or more nodes and generally experience greater losses due to scattering and interaction with waveguide imperfections.
The number of modes that can propagate within a waveguide is dependent on the frequency of the acoustic wave and the waveguide dimensions. At lower frequencies, only the fundamental mode may be supported, resulting in single-mode propagation. As frequency increases or waveguide dimensions enlarge, higher-order modes become permissible, leading to multi-mode propagation. While multi-mode propagation can increase the information carrying capacity in some communication applications, it can also introduce complexities such as modal dispersion and intermodal interference, which can degrade signal quality.
The specific shapes and characteristics of the modes are determined by solving the acoustic wave equation subject to the boundary conditions at the waveguide interfaces. For simple geometries like planar or cylindrical waveguides, analytical solutions can be derived. For more complex structures, numerical methods such as finite element analysis or boundary element methods are employed to calculate the modal profiles and propagation constants.
Dispersion and Group Velocity
Dispersion is a phenomenon where the propagation velocity of a wave depends on its frequency. In acoustic waveguides, dispersion arises due to the interaction of the acoustic wave with the waveguide boundaries and the frequency-dependent nature of the material properties. Dispersion can be categorized into two main types: modal dispersion and material dispersion.
Modal dispersion, also known as waveguide dispersion, occurs in multi-mode waveguides because different modes propagate with different velocities. This means that if a pulse of sound composed of multiple modes is launched into the waveguide, the modes will arrive at the output at different times, causing the pulse to broaden. This effect can limit the bandwidth of acoustic waveguides used for communication or sensing applications.
Material dispersion arises from the frequency dependence of the acoustic properties of the materials comprising the waveguide, such as the speed of sound and density. Most materials exhibit some degree of frequency dependence in their acoustic properties, especially at higher frequencies. This frequency dependence can lead to variations in the propagation velocity of different frequency components within a signal, causing pulse broadening even in single-mode waveguides.
The group velocity is a crucial concept in understanding dispersion. It represents the velocity at which the envelope of a wave packet, or signal, propagates. In dispersive media, the group velocity is different from the phase velocity, which is the velocity of a single frequency component of the wave. The group velocity is given by the derivative of the angular frequency with respect to the wavenumber. In waveguides, the group velocity is mode-dependent and frequency-dependent, reflecting the combined effects of modal and material dispersion.
Understanding and managing dispersion is critical in designing acoustic waveguide systems. In some applications, such as long-distance underwater communication, dispersion compensation techniques are employed to minimize pulse broadening and maintain signal integrity. In other applications, such as pulse compression in ultrasonic imaging, dispersive properties of waveguides are intentionally exploited to manipulate and shape acoustic pulses.
Materials and Structures for Acoustic Waveguides
The selection of materials and the design of the waveguide structure are paramount in determining the performance characteristics of acoustic waveguides. The key material properties are acoustic impedance, attenuation, and the speed of sound. The structural design involves shaping the waveguide to achieve desired mode confinement, bandwidth, and operational frequency range.
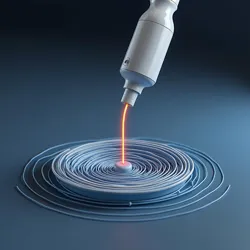
Solid Waveguides
Solid materials are frequently used in acoustic waveguiding, particularly for applications involving high frequencies and short wavelengths, such as ultrasonic imaging and non-destructive testing. Common solid waveguide materials include metals, glasses, ceramics, and polymers. The choice of material depends on the specific application requirements, including frequency range, bandwidth, acoustic impedance matching to the surrounding medium, and mechanical properties.
Metallic waveguides, such as aluminum or steel rods or plates, are robust and exhibit relatively low acoustic attenuation at lower frequencies. They are often used for long-range acoustic transmission in structural health monitoring and seismic exploration. Glass waveguides offer good acoustic transmission characteristics and are used in some ultrasonic delay lines and sensors. Ceramic materials, like piezoelectric ceramics, can be used to create active waveguides that can generate and detect acoustic waves, enabling integrated acoustic devices.
Polymeric waveguides, made from materials like polymethylmethacrylate (PMMA) or silicone rubber, are flexible, lightweight, and can be easily fabricated into complex shapes. They are suitable for applications requiring conformable or biocompatible acoustic waveguides, such as wearable sensors or medical ultrasound probes. However, polymers generally exhibit higher acoustic attenuation compared to metals and glasses, especially at higher frequencies.
The structure of solid waveguides can vary widely. Simple geometries include rods, plates, and fibers. More complex structures, such as phononic crystals and metamaterials, can be engineered to achieve tailored acoustic wave propagation characteristics, including negative refraction, cloaking, and subwavelength focusing. Solid waveguides are often used in contact-based applications where the waveguide is physically coupled to the object under investigation or the medium through which sound needs to be transmitted.
Fluid Waveguides
Fluid waveguides utilize the contrast in acoustic impedance between fluids and solid boundaries or between different fluids to guide acoustic waves. Water, air, and various oils and gases can serve as fluid waveguide media. Fluid waveguides are particularly relevant in underwater acoustics, atmospheric acoustics, and medical applications where the propagation medium is primarily fluid.
Underwater acoustic channels in the ocean are natural examples of fluid waveguides. The ocean's sound speed profile, which varies with depth due to temperature and pressure gradients, creates waveguide effects. The deep sound channel, or SOFAR channel (Sound Fixing and Ranging channel), is a well-known example where sound waves are trapped and guided over long distances due to refraction caused by the sound speed minimum at a certain depth. This phenomenon is exploited in underwater communication and sonar systems.
Air ducts and pipes can act as fluid waveguides for airborne sound. The rigid walls of the duct provide impedance contrast with the air, causing reflection and guiding of sound waves along the duct. This principle is utilized in musical instruments like flutes and organ pipes, as well as in ventilation systems and acoustic liners for noise reduction.
In medical applications, fluid-filled catheters or endoscopes can serve as waveguides for delivering and receiving ultrasound energy within the body. The fluid within the catheter provides an acoustic pathway, while the catheter walls provide confinement. This technique is used in intravascular ultrasound (IVUS) and endoscopic ultrasound for imaging internal organs and blood vessels.
Phononic Crystals
Phononic crystals are artificially engineered periodic structures that exhibit unique acoustic wave propagation properties. They are analogous to photonic crystals in optics but designed to manipulate phonons, the quanta of lattice vibrations, or acoustic waves. Phononic crystals are composed of periodic arrangements of materials with differing acoustic impedances. This periodicity creates phononic band gaps, frequency ranges where acoustic wave propagation is forbidden.
Within the band gaps, acoustic waves incident on the phononic crystal are strongly reflected, regardless of the angle of incidence. This property can be used to create highly efficient acoustic reflectors and waveguides. By introducing defects or modifying the periodicity of the phononic crystal, it is possible to create localized modes within the band gap, allowing for the design of resonant cavities, filters, and waveguide bends.
Phononic crystals can be fabricated in various dimensions, including one-dimensional (periodic multilayers), two-dimensional (periodic arrays of rods or holes), and three-dimensional structures. The dimensions and periodicity of the structure are typically on the order of the acoustic wavelength at the desired operating frequency. Phononic crystals offer unprecedented control over acoustic wave propagation and have potential applications in acoustic cloaking, focusing, sensing, and advanced signal processing.
One interesting type of phononic crystal waveguide is based on topological phononics. These structures exhibit robust waveguiding properties that are protected from backscattering and imperfections. Topological acoustic waveguides are designed based on concepts from topological insulators in condensed matter physics. They offer the potential for highly reliable and efficient acoustic wave transmission, even in the presence of defects or disorder.
Applications of Acoustic Waveguiding
Acoustic waveguiding principles are leveraged in a wide array of applications, spanning medical diagnostics, industrial testing, communication technologies, and sensing systems. The ability to precisely control and direct acoustic energy has opened up new possibilities in these diverse fields.
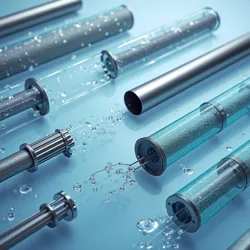
Medical Ultrasonography
In medical ultrasonography, acoustic waveguides play a crucial role in delivering and receiving high-frequency sound waves for imaging internal organs and tissues. Ultrasound probes often incorporate acoustic lenses and waveguides to focus the acoustic beam and improve image resolution. Arrays of small waveguide elements are used in phased array transducers to steer and focus the ultrasound beam electronically, enabling real-time volumetric imaging.
Intracavitary ultrasound probes, such as transesophageal echocardiography (TEE) probes and endorectal ultrasound probes, utilize flexible acoustic waveguides to navigate through body cavities and reach target organs. These waveguides must be biocompatible, minimally invasive, and capable of transmitting ultrasound with minimal attenuation. Miniaturized ultrasound probes incorporating acoustic waveguides are also being developed for intravascular imaging and minimally invasive surgical guidance.
Acoustic waveguiding is also being explored for therapeutic ultrasound applications, such as high-intensity focused ultrasound (HIFU) for targeted tissue ablation and drug delivery. Waveguides can be designed to precisely focus high-intensity ultrasound beams onto tumors or other target tissues deep within the body, minimizing damage to surrounding healthy tissue. Furthermore, waveguide-based ultrasound applicators can be used for localized drug delivery, enhancing the efficacy of chemotherapy or gene therapy.
Non-Destructive Testing
Non-destructive testing (NDT) relies heavily on acoustic waveguiding techniques to inspect materials and structures for defects without causing damage. Ultrasonic testing (UT) is a widely used NDT method that utilizes acoustic waves to detect cracks, voids, inclusions, and other flaws in materials. Acoustic waveguides, in the form of guided wave probes, are employed to transmit ultrasonic waves along the surface or within the volume of the component under inspection.
Guided wave testing, also known as long-range ultrasonic testing (LRUT), is particularly effective for inspecting pipelines, rails, and large structures. Guided waves, such as Lamb waves and shear horizontal waves, can propagate over long distances along the waveguide structure, allowing for rapid and cost-effective inspection of extended areas from a single access point. Waveguide probes are designed to excite and receive specific guided wave modes that are sensitive to different types of defects and structural features.
Acoustic waveguides are also used in ultrasonic thickness gauging, corrosion mapping, and material characterization. Specialized waveguide probes can be designed to operate in harsh environments, such as high temperatures or corrosive atmospheres, enabling NDT in challenging industrial settings. The use of phononic crystal waveguides in NDT is also being explored to enhance defect detection sensitivity and spatial resolution.
Acoustic Sensors
Acoustic waveguides form the basis of various types of acoustic sensors used for detecting physical, chemical, and biological parameters. Waveguide-based acoustic sensors offer high sensitivity, miniaturization potential, and real-time monitoring capabilities.
Surface acoustic wave (SAW) sensors and bulk acoustic wave (BAW) sensors are prominent examples of waveguide-based acoustic sensors. SAW sensors utilize acoustic waves propagating along the surface of a piezoelectric substrate, while BAW sensors employ acoustic waves propagating through the bulk of the substrate. Changes in the properties of the medium in contact with the waveguide, such as mass loading, viscosity, or elasticity, alter the propagation characteristics of the acoustic waves, which can be detected and correlated to the sensed parameter.
Waveguide-based acoustic sensors are used for a wide range of applications, including gas sensing, liquid sensing, pressure sensing, temperature sensing, and biosensing. For example, SAW sensors are used in automotive airbag deployment systems, tire pressure monitoring systems, and wireless communication devices. BAW sensors, particularly quartz crystal microbalances (QCMs), are widely used for mass sensing in chemical analysis and biological assays.
Acoustic waveguide sensors are also being developed for more specialized applications, such as underwater acoustic sensing, structural health monitoring, and environmental monitoring. Fiber optic acoustic sensors, where acoustic waves modulate the light propagating in an optical fiber, offer advantages for remote sensing and distributed sensing networks.
Nautophone Communications
A novel application of acoustic waveguiding is in Nautophone Communications, a method of underwater communication that utilizes specialized acoustic waveguides to transmit information over significant distances with enhanced security and efficiency. Unlike traditional underwater acoustic communication systems that rely on omnidirectional or directional transducers radiating sound directly into the water, nautophone systems employ submerged acoustic cables or waveguide structures to confine and guide acoustic signals.
These waveguides, often constructed from materials with carefully engineered acoustic impedance properties, can be deployed along the seabed or suspended within the water column. They effectively channel the acoustic energy, minimizing signal dissipation and reducing interference from ambient noise and other acoustic sources. This guided wave approach allows for lower transmission power, improved signal-to-noise ratios, and greater communication range compared to conventional underwater acoustic communication methods.
Furthermore, the confined nature of acoustic wave propagation within the waveguides enhances communication security. Eavesdropping becomes significantly more challenging as the acoustic signals are primarily contained within the waveguide structure, making it difficult for unauthorized parties to intercept or decode the transmitted information. This aspect is particularly valuable for sensitive underwater communication applications, such as military or strategic communications.
Nautophone communication systems can be designed for various underwater communication scenarios, including point-to-point links between submerged stations, communication with underwater vehicles, and seabed-based sensor networks. The waveguides can be tailored to specific frequency bands and operational environments to optimize performance and minimize losses. The development of advanced acoustic materials and waveguide fabrication techniques is further enhancing the capabilities and practicality of nautophone communication systems, paving the way for more robust and secure underwater communication networks.
Historical and Theoretical Developments
The principles of acoustic waveguiding have been recognized and applied for centuries, albeit often implicitly, in various natural phenomena and human inventions. The formal theoretical framework and systematic engineering of acoustic waveguides emerged primarily in the 20th century, paralleling developments in electromagnetic and optical waveguiding.
Early Observations
Natural examples of acoustic waveguiding have been observed throughout history. The phenomenon of sound propagation over long distances in the ocean's SOFAR channel has been known to mariners for generations, even before its scientific explanation. Similarly, the guiding of seismic waves within the Earth's crust and mantle, known as the wave guide effect in seismology, has been recognized through the study of earthquake waves.
In musical instruments, the principles of acoustic waveguiding have been intuitively employed for centuries. Wind instruments, such as flutes, clarinets, and organ pipes, rely on air columns confined within tubes or ducts to produce and shape musical tones. The geometry and dimensions of these waveguides determine the resonant frequencies and tonal characteristics of the instruments. Stringed instruments, like violins and guitars, also utilize the resonant properties of the instrument body, which acts as a complex acoustic waveguide, to amplify and project sound.
Early forms of acoustic communication, such as speaking tubes used in ships and buildings, also employed waveguide principles to transmit speech over distances. These tubes, acting as simple acoustic waveguides, confined the sound waves and reduced sound attenuation compared to open-air transmission.
Mathematical Frameworks
The formal theoretical framework for acoustic waveguiding began to develop in the early to mid-20th century, building upon the classical wave equation and boundary value problem formulations. The work of Lord Rayleigh on sound theory in the late 19th and early 20th centuries provided foundational principles for understanding wave propagation and reflection phenomena relevant to waveguiding.
The development of electromagnetic waveguide theory in the context of radio and microwave engineering in the 1930s and 1940s provided a strong analogy and mathematical toolbox for acoustic waveguiding research. Solutions to the Helmholtz equation, a frequency-domain form of the wave equation, were adapted to analyze acoustic wave propagation in various waveguide geometries, such as planar slabs, cylinders, and rectangular ducts.
The modal theory of waveguides, which describes wave propagation in terms of a superposition of eigenmodes, became a central concept. Mathematical techniques for solving eigenvalue problems and determining modal propagation constants were developed and applied to acoustic waveguides. The concepts of group velocity, dispersion, and modal attenuation were also rigorously formulated and analyzed.
The development of numerical methods, such as finite element analysis and boundary element methods, in the latter half of the 20th century enabled the analysis of more complex waveguide structures and materials. These numerical tools became essential for designing and optimizing acoustic waveguides for specific applications.
Modern Advances
Modern advances in acoustic waveguiding are driven by advancements in materials science, microfabrication techniques, and computational modeling. The development of novel acoustic materials, such as phononic crystals, metamaterials, and topological acoustic materials, has opened up new possibilities for manipulating acoustic waves with unprecedented control.
Microfabrication techniques, such as micromachining, thin film deposition, and nanoimprint lithography, have enabled the fabrication of miniaturized acoustic waveguides and devices for microacoustic and nanoacoustic applications. These techniques are crucial for creating integrated acoustic sensors, actuators, and communication components.
Computational modeling and simulation tools have become increasingly sophisticated, allowing for the design and optimization of complex waveguide structures and devices with high accuracy and efficiency. Inverse design methods, using optimization algorithms and machine learning, are being explored to automatically design acoustic waveguides with desired performance characteristics.
Current research in acoustic waveguiding is focused on areas such as:
- High-frequency acoustic waveguides: Exploring waveguides operating at gigahertz and terahertz frequencies for advanced imaging, sensing, and communication applications.
- Metamaterial and phononic crystal waveguides: Developing waveguides with tailored dispersion, negative refraction, cloaking, and topological protection properties.
- Integrated acoustic waveguides: Integrating acoustic waveguides with electronic and optical components for creating multi-functional devices and systems.
- Biocompatible and flexible acoustic waveguides: Developing waveguides for medical implants, wearable sensors, and bioacoustic applications.
- Nonlinear acoustic waveguides: Investigating nonlinear wave propagation phenomena in waveguides for signal processing and energy harvesting.
These ongoing research efforts promise to further expand the capabilities and applications of acoustic waveguiding, contributing to advancements in various fields of science and technology.